Biomass
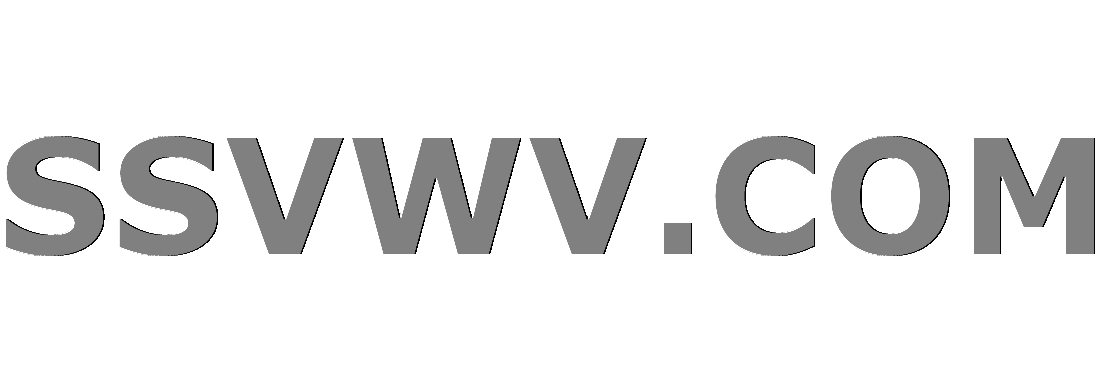
Multi tool use

Wood pellets
Biomass is plant or animal material used for energy production, heat production, or in various industrial processes as raw material for a range of products.[1] It can be purposely grown energy crops (e.g. miscanthus, switchgrass), wood or forest residues, waste from food crops (wheat straw, bagasse), horticulture (yard waste), food processing (corn cobs), animal farming (manure, rich in nitrogen and phosphorus), or human waste from sewage plants.[2]
Burning plant-derived biomass releases CO2, but it has still been classified as a renewable energy source in the EU and UN legal frameworks because photosynthesis cycles the CO2 back into new crops. In some cases, this recycling of CO2 from plants to atmosphere and back into plants can even be CO2 negative, as a relatively large portion of the CO2 is moved to the soil during each cycle.
Cofiring with biomass has increased in coal power plants, because it makes it possible to release less CO2 without the cost assosicated with building new infrastructure. Co-firing is not without issues however, often an upgrade of the biomass is beneficiary. Upgrading to higher grade fuels can be achieved by different methods, broadly classified as thermal, chemical, or biochemical (see below).
.mw-parser-output .quotebox{background-color:#F9F9F9;border:1px solid #aaa;box-sizing:border-box;padding:10px;font-size:88%;max-width:100%}.mw-parser-output .quotebox.floatleft{margin:0.5em 1.4em 0.8em 0}.mw-parser-output .quotebox.floatright{margin:0.5em 0 0.8em 1.4em}.mw-parser-output .quotebox.centered{margin:0.5em auto 0.8em auto}.mw-parser-output .quotebox.floatleft p,.mw-parser-output .quotebox.floatright p{font-style:inherit}.mw-parser-output .quotebox-title{background-color:#F9F9F9;text-align:center;font-size:larger;font-weight:bold}.mw-parser-output .quotebox-quote.quoted:before{font-family:"Times New Roman",serif;font-weight:bold;font-size:large;color:gray;content:" “ ";vertical-align:-45%;line-height:0}.mw-parser-output .quotebox-quote.quoted:after{font-family:"Times New Roman",serif;font-weight:bold;font-size:large;color:gray;content:" ” ";line-height:0}.mw-parser-output .quotebox .left-aligned{text-align:left}.mw-parser-output .quotebox .right-aligned{text-align:right}.mw-parser-output .quotebox .center-aligned{text-align:center}.mw-parser-output .quotebox cite{display:block;font-style:normal}@media screen and (max-width:360px){.mw-parser-output .quotebox{min-width:100%;margin:0 0 0.8em!important;float:none!important}}
IUPAC definition
Biomass: Material produced by the growth of microorganisms, plants or animals. [3]
Contents
1 Biomass feedstocks
2 Biomass conversion
2.1 Thermal conversions
2.2 Chemical conversion
2.3 Biochemical conversion
2.4 Electrochemical conversion
3 Environmental impact
4 See also
5 References
6 External links
Biomass feedstocks

Biomass plant in Scotland.
Wood waste outside biomass power plant.

Bagasse is the remaining waste after sugar canes have been crushed to extract their juice.
Miscanthus x giganteus energy crop, Germany.
Historically, humans have harnessed biomass-derived energy since the time when people began burning wood fuel.[4] Even in 2019, biomass is the only source of fuel for domestic use in many developing countries. All biomass is biologically-produced matter based in carbon, hydrogen and oxygen. The estimated biomass production in the world is approximately 100 billion metric tons of carbon per year, about half in the ocean and half on land.[5]
Wood and residues from wood, for instance spruce, birch, eucalyptus, willow, oil palm, remains the largest biomass energy source today.[4] It is used directly as a fuel or processed into pellet fuel or other forms of fuels. Biomass also includes plant or animal matter that can be converted into fuel, fibers or industrial chemicals. There are numerous types of plants, including corn, switchgrass, miscanthus, hemp, sorghum, sugarcane, and bamboo.[6] The main waste energy feedstocks are wood waste, agricultural waste, municipal solid waste, manufacturing waste, and landfill gas. Sewage sludge is another source of biomass. There is ongoing research involving algae or algae-derived biomass.[7] Other biomass feedstocks are enzymes or bacteria from various sources, grown in cell cultures or hydroponics.[8][9]
Based on the source of biomass, biofuels are classified broadly into two major categories:
First-generation biofuels are derived from food sources, such as sugarcane and corn starch. Sugars present in this biomass are fermented to produce bioethanol, an alcohol fuel which serve as an additive to gasoline, or in a fuel cell to produce electricity.[10]
Second-generation biofuels utilize non-food-based biomass sources such as perennial energy crops (low input crops), and agricultural/municipal waste. There is huge potential for second generation biofuels but the resources are currently under-utilized.[11]
Biomass conversion
Thermal conversions

Straw bales
Thermal conversion processes use heat as the dominant mechanism to upgrade biomass into a better and more practical fuel. The basic alternatives are torrefaction, pyrolysis, and gasification, these are separated principally by the extent to which the chemical reactions involved are allowed to proceed (mainly controlled by the availability of oxygen and conversion temperature).[12]
There are other less common, more experimental or proprietary thermal processes that may offer benefits, such as hydrothermal upgrading.[citation needed] Some have been developed for use on high moisture content biomass, including aqueous slurries, and allow them to be converted into more convenient forms.
Chemical conversion
A range of chemical processes may be used to convert biomass into other forms, such as to produce a fuel that is more practical to store, transport and use, or to exploit some property of the process itself. Many of these processes are based in large part on similar coal-based processes, such as the Fischer-Tropsch synthesis.[13]
Biomass can be converted into multiple commodity chemicals.[14]
Biochemical conversion
As biomass is a natural material, many highly efficient biochemical processes have developed in nature to break down the molecules of which biomass is composed, and many of these biochemical conversion processes can be harnessed. In most cases, microorganisms are used to perform the conversion process: anaerobic digestion, fermentation, and composting.[15]
Glycoside hydrolases are the enzymes involved in the degradation of the major fraction of biomass, such as polysaccharides present in starch and lignocellulose. Thermostable variants are gaining increasing roles as catalysts in biorefining applications, since recalcitrant biomass often needs thermal treatment for more efficient degradation.[16]
Electrochemical conversion
Biomass can be directly converted to electrical energy via electrochemical (electrocatalytic) oxidation of the material. This can be performed directly in a direct carbon fuel cell,[17] direct liquid fuel cells such as direct ethanol fuel cell, a direct methanol fuel cell, a direct formic acid fuel cell, a L-ascorbic Acid Fuel Cell (vitamin C fuel cell),[18] and a microbial fuel cell.[19] The fuel can also be consumed indirectly via a fuel cell system containing a reformer which converts the biomass into a mixture of CO and H2 before it is consumed in the fuel cell.[20]
Environmental impact
On combustion, the carbon from biomass is released into the atmosphere as carbon dioxide (CO2). After a few months, or years, or decades, the CO2 has been absorbed back by growing plants or trees. However, the carbon storage capacity of forests may be reduced overall if destructive forestry techniques are employed.[21][22][23][24]
All biomass crops sequester carbon. For example, soil organic carbon has been observed to be greater below switchgrass crops than under cultivated cropland, especially at depths below 30 cm (12 in).[25] For Miscanthus x giganteus, McCalmont et al. found accumulation rates ranging from 0.42 to 3.8 tonnes per hectare per year,
[26]
with a mean accumulation rate of 1.84 tonne (0.74 tonnes per acre per year),
[27]
or 20% of total harvested carbon per year.
[28] The grass sequesters carbon in its continually increasing root biomass, toghether with carbon input from fallen leaves. Typically, perennial crops sequester significantly more carbon than annual crops due to greater non-harvested living biomass (roots and residues), both living and dead, built up over years, and less soil disruption in cultivation.

GHG / CO2 / carbon negativity for Miscanthus x giganteus production pathways.

Relationship between above-ground yield (diagonal lines), soil organic carbon (X axis), and soil's potential for successful/unsuccessful carbon sequestration (Y axis). Basically, the higher the yield, the more land is usable as a GHG mitigation tool (including relatively carbon rich land.)
The simple proposal that biomass is carbon-neutral put forward in the early 1990s has been superseded by the more nuanced proposal that for a particular bioenergy project to be carbon neutral, the total carbon sequestered by a bioenergy crop's root system must compensate for all the emissions from the related, aboveground bioenergy project. This includes any emissions caused by direct or indirect land use change. Many first generation bioenergy projects are not carbon neutral given these demands. Some have even higher total GHG emissions than some fossil based alternatives.[29][30][31]
Transport fuels might be worse than solid fuels in this regard.
[32]
Some are carbon neutral or even negative, though, especially perennial crops. The amount of carbon sequestrated and the amount of GHG (greenhouse gases) emitted will determine if the total GHG life cycle cost of a bio-energy project is positive, neutral or negative. Whitaker et al. estimates that for Miscanthus x giganteus, GHG neutrality and even negativity is within reach. A carbon negative life cycle is possible if the total below-ground carbon accumulation more than compensates for the above-ground total life-cycle GHG emissions.
The graphic on the right displays two CO2 negative Miscanthus x giganteus production pathways, represented in gram CO2-equivalents per megajoule. The yellow diamonds represent mean values.
[33]
Successful sequestration is dependent on planting sites, as the best soils for sequestration are those that are currently low in carbon. The varied results displayed in the graph highlights this fact.
[34]
For the UK, successful sequestration is expected for arable land over most of England and Wales, with unsucessful sequestration expected in parts of Scotland, due to already carbon rich soils (existing woodland) plus lower yields. Soils already rich in carbon includes peatland and mature forest. Grassland can also be carbon rich, however Milner et al. argues that the most successful carbon sequestration in the UK takes place below improved grasslands.
[35]
The bottom graphic displays the estimated yield necessary to compensate for the disturbance caused by planting plus lifecycle GHG-emissions for the related above-ground operation.
Forest-based biomass projects has received criticism for ineffective GHG mitigation from a number of environmental organizations, including Greenpeace and the Natural Resources Defense Council. Environmental groups also argue that it might take decades for the carbon released by burning biomass to be recaptured by new trees. Biomass burning produces air pollution in the form of carbon monoxide, volatile organic compounds, particulates and other pollutants.[36][37][38] In 2009 a Swedish study of the giant brown haze that periodically covers large areas in South Asia determined that two thirds of it had been principally produced by residential cooking and agricultural burning, and one third by fossil-fuel burning.[39] Use of wood biomass as an industrial fuel produce fewer particulates and other pollutants than the burning seen in wildfires or open field fires.[40]
See also
Part of a series on |
Renewable energy |
---|
|
|
- Biochar
- Bioenergy
- Biofact (biology)
- Biofuel
- Biomass (ecology)
- Biomass gasification
- Biomass heating systems
- Biomass to liquid
- Bioproduct
- Biorefinery
- Carbon
- European Biomass Association
- Carbon footprint
- Cow dung
- Energy crop
- Energy forestry
- Firewood
- Microgeneration
Microbial electrolysis cell generates hydrogen or methane- Pellet fuel
- Permaculture
- Thermal mass
Wood fuel (a traditional biomass fuel)- Woodchips
References
^ Ur-Rehman, S; Mushtaq, Z; Zahoor, T; Jamil, A; Murtaza, MA (2015). "Xylitol: a review on bioproduction, application, health benefits, and related safety issues". Critical Reviews in Food Science and Nutrition. 55 (11): 1514–28. doi:10.1080/10408398.2012.702288. PMID 24915309..mw-parser-output cite.citation{font-style:inherit}.mw-parser-output .citation q{quotes:"""""""'""'"}.mw-parser-output .citation .cs1-lock-free a{background:url("//upload.wikimedia.org/wikipedia/commons/thumb/6/65/Lock-green.svg/9px-Lock-green.svg.png")no-repeat;background-position:right .1em center}.mw-parser-output .citation .cs1-lock-limited a,.mw-parser-output .citation .cs1-lock-registration a{background:url("//upload.wikimedia.org/wikipedia/commons/thumb/d/d6/Lock-gray-alt-2.svg/9px-Lock-gray-alt-2.svg.png")no-repeat;background-position:right .1em center}.mw-parser-output .citation .cs1-lock-subscription a{background:url("//upload.wikimedia.org/wikipedia/commons/thumb/a/aa/Lock-red-alt-2.svg/9px-Lock-red-alt-2.svg.png")no-repeat;background-position:right .1em center}.mw-parser-output .cs1-subscription,.mw-parser-output .cs1-registration{color:#555}.mw-parser-output .cs1-subscription span,.mw-parser-output .cs1-registration span{border-bottom:1px dotted;cursor:help}.mw-parser-output .cs1-ws-icon a{background:url("//upload.wikimedia.org/wikipedia/commons/thumb/4/4c/Wikisource-logo.svg/12px-Wikisource-logo.svg.png")no-repeat;background-position:right .1em center}.mw-parser-output code.cs1-code{color:inherit;background:inherit;border:inherit;padding:inherit}.mw-parser-output .cs1-hidden-error{display:none;font-size:100%}.mw-parser-output .cs1-visible-error{font-size:100%}.mw-parser-output .cs1-maint{display:none;color:#33aa33;margin-left:0.3em}.mw-parser-output .cs1-subscription,.mw-parser-output .cs1-registration,.mw-parser-output .cs1-format{font-size:95%}.mw-parser-output .cs1-kern-left,.mw-parser-output .cs1-kern-wl-left{padding-left:0.2em}.mw-parser-output .cs1-kern-right,.mw-parser-output .cs1-kern-wl-right{padding-right:0.2em}
^ "Biomass - Energy Explained, Your Guide To Understanding Energy". U.S. Energy Information Administration. June 21, 2018.
^ Nagel, B.; Dellweg, H.; Gierasch, L. M. (1 January 1992). "Glossary for chemists of terms used in biotechnology (IUPAC Recommendations 1992)". Pure and Applied Chemistry. 64 (1): 143–168. doi:10.1351/pac199264010143.
^ ab [1] Retrieved on 2012-04-12.
^ Field, C. B.; Behrenfeld, M. J.; Randerson, J. T.; Falkowski, P. (1998). "Primary Production of the Biosphere: Integrating Terrestrial and Oceanic Components" (PDF). Science (Submitted manuscript). 281 (5374): 237–240. Bibcode:1998Sci...281..237F. doi:10.1126/science.281.5374.237. PMID 9657713.
^ Darby, Thomas. "What Is Biomass Renewable Energy". Real World Energy. Archived from the original on 2014-06-08. Retrieved 12 June 2014.
^ Randor Radakovits; Robert E. Jinkerson; Al Darzins; Matthew C. Posewitz1 (2010). "Genetic Engineering of Algae for Enhanced Biofuel Production". Eukaryotic Cell. 9 (4): 486–501. doi:10.1128/EC.00364-09. PMC 2863401. PMID 20139239.
^ Biomass-to-Fuel Conversion (Princeton University USA)
^ The Nocera lab
^ Martin, Marshall A. (1 November 2010). "First generation biofuels compete". New Biotechnology. 27 (5): 596–608. doi:10.1016/j.nbt.2010.06.010. PMID 20601265.
^ Kosinkova, Jana; Doshi, Amar; Maire, Juliette; Ristovski, Zoran; Brown, Richard; Rainey, Thomas (September 2015). "Measuring the regional availability of biomass for biofuels and the potential for microalgae". Renewable and Sustainable Energy Reviews. 49: 1271–1285. doi:10.1016/j.rser.2015.04.084.
^ Akhtar, A., Krepl, V., & Ivanova, T. (2018). A Combined Overview of Combustion, Pyrolysis, and Gasification of Biomass. Energy & Fuels, 32(7), 7294–7318.
^ Liu, G., E. D. Larson, R. H. Williams, T. G. Kreutz and X. Guo (2011). "Making fischer-tropsch fuels and electricity from coal and biomass: Performance and cost analysis." Energy & Fuels 25: 415–437.
^ Conversion technologies. Biomassenergycentre.org.uk. Retrieved on 2012-02-28.
^ "Biochemical Conversion of Biomass". BioEnergy Consult. 2014-05-29. Retrieved 2016-10-18.
^ Linares-Pastén, J. A.; Andersson, M; Nordberg karlsson, E (2014). "Thermostable glycoside hydrolases in biorefinery technologies" (PDF). Current Biotechnology. 3 (1): 26–44. doi:10.2174/22115501113026660041.
^ Munnings, C.; Kulkarni, A.; Giddey, S.; Badwal, S.P.S. (August 2014). "Biomass to power conversion in a direct carbon fuel cell". International Journal of Hydrogen Energy. 39 (23): 12377–12385. doi:10.1016/j.ijhydene.2014.03.255.
^ Kim, Ye Eun (17 May 2011). "Surface Modifications of a Carbon Anode Catalyst by Control of Functional Groups for Vitamin C Fuel Cells". Electrocatalysis. 2 (3): 200–206. doi:10.1007/s12678-011-0055-0.
^ Knight, Chris (2013). "Chapter 6 – Application of Microbial Fuel Cells to Power Sensor Networks for Ecological Monitoring". Wireless Sensor Networks and Ecological Monitoring. Smart Sensors, Measurement and Instrumentation. 3. pp. 151–178. doi:10.1007/978-3-642-36365-8_6. ISBN 978-3-642-36364-1.
^ Badwal, Sukhvinder P. S.; Giddey, Sarbjit S.; Munnings, Christopher; Bhatt, Anand I.; Hollenkamp, Anthony F. (24 September 2014). "Emerging electrochemical energy conversion and storage technologies (open access)". Frontiers in Chemistry. 2: 79. Bibcode:2014FrCh....2...79B. doi:10.3389/fchem.2014.00079. PMC 4174133. PMID 25309898.
^ Prasad, Ram. "SUSTAINABLE FOREST MANAGEMENT FOR DRY FORESTS OF SOUTH ASIA". Food and Agriculture Organization of the United Nations. Retrieved 11 August 2010.
^ "Treetrouble: Testimonies on the Negative Impact of Large-scale Tree Plantations prepared for the sixth Conference of the Parties of the Framework Convention on Climate Change". Friends of the Earth International. Archived from the original on 26 July 2011. Retrieved 11 August 2010.
^ Laiho, Raija; Sanchez, Felipe; Tiarks, Allan; Dougherty, Phillip M.; Trettin, Carl C. "Impacts of intensive forestry on early rotation trends in site carbon pools in the southeastern US". United States Department of Agriculture. Retrieved 11 August 2010.
^ "THE FINANCIAL AND INSTITUTIONAL FEASIBILITY OF SUSTAINABLE FOREST MANAGEMENT". Food and Agriculture Organization of the United Nations. Retrieved 11 August 2010.
^ Soil Carbon under Switchgrass Stands and Cultivated Cropland (Interpretive Summary and Technical Abstract). USDA Agricultural Research Service, April 1, 2005
^ «[…] it seems likely that arable land converted to Miscanthus will sequester soil carbon; of the 14 comparisons, 11 showed overall increases in SOC over their total sample depths with suggested accumulation rates ranging from 0.42 to 3.8 Mg C ha-1 yr-1. Only three arable comparisons showed lower SOC stocks under Miscanthus, and these suggested insignificant losses between 0.1 and 0.26 Mg ha-1 yr-1.»
McCalmont, J. P., Hastings, A. , McNamara, N. P., Richter, G. M., Robson, P. , Donnison, I. S. and Clifton‐Brown, J. (2017), Environmental costs and benefits of growing Miscanthus for bioenergy in the UK. GCB Bioenergy, 9, page 493. https://doi.org/10.1111/gcbb.12294This article incorporates text available under the CC BY 4.0 license. (The CC BY 4.0 licence means that everyone have the right to reuse the text that is quoted here, or other parts of the original article itself, if they credit the authors. More info: https://en.wikipedia.org/wiki/Creative_Commons_license)
^ «The correlation between plantation age and SOC can be seen in Fig. 6, […] the trendline suggests a net accumulation rate of 1.84 Mg C ha-1 yr-1 with similar levels to grassland at equilibrium.»
McCalmont, J. P., Hastings, A. , McNamara, N. P., Richter, G. M., Robson, P. , Donnison, I. S. and Clifton‐Brown, J. (2017), Environmental costs and benefits of growing Miscanthus for bioenergy in the UK. GCB Bioenergy, 9, page 496. https://doi.org/10.1111/gcbb.12294This article incorporates text available under the CC BY 4.0 license. (The CC BY 4.0 licence means that everyone have the right to reuse the text that is quoted here, or other parts of the original article itself, if they credit the authors. More info: https://en.wikipedia.org/wiki/Creative_Commons_license)
^ Given the EU average yield of 18.8 tonnes dry matter per hectare per year (see Clifton-Brown, above), and 48% carbon content (see Kahle et al,, above).
^ «The environmental costs and benefits of bioenergy have been the subject of significant debate, particularly for first‐generation biofuels produced from food (e.g. grain and oil seed). Studies have reported life‐cycle GHG savings ranging from an 86% reduction to a 93% increase in GHG emissions compared with fossil fuels (Searchinger et al., 2008; Davis et al., 2009; Liska et al., 2009; Whitaker et al., 2010). In addition, concerns have been raised that N2O emissions from biofuel feedstock cultivation could have been underestimated (Crutzen et al., 2008; Smith & Searchinger, 2012) and that expansion of feedstock cultivation on agricultural land might displace food production onto land with high carbon stocks or high conservation value (i.e. iLUC) creating a carbon debt which could take decades to repay (Fargione et al., 2008). Other studies have shown that direct nitrogen‐related emissions from annual crop feedstocks can be mitigated through optimized management practices (Davis et al., 2013) or that payback times are less significant than proposed (Mello et al., 2014). However, there are still significant concerns over the impacts of iLUC, despite policy developments aimed at reducing the risk of iLUC occurring (Ahlgren & Di Lucia, 2014; Del Grosso et al., 2014).»
Whitaker, J. , Field, J. L., Bernacchi, C. J., Cerri, C. E., Ceulemans, R. , Davies, C. A., DeLucia, E. H., Donnison, I. S., McCalmont, J. P., Paustian, K. , Rowe, R. L., Smith, P. , Thornley, P. and McNamara, N. P. (2018), Consensus, uncertainties and challenges for perennial bioenergy crops and land use. GCB Bioenergy, 10: 150-164. https://doi.org/10.1111/gcbb.12488This article incorporates text available under the CC BY 4.0 license. (The CC BY 4.0 licence means that everyone have the right to reuse the text that is quoted here, or other parts of the original article itself, if they credit the authors. More info: https://en.wikipedia.org/wiki/Creative_Commons_license)
^ «The impact of growing bioenergy and biofuel feedstock crops has been of particular concern, with some suggesting the greenhouse gas (GHG) balance of food crops used for ethanol and biodiesel may be no better or worse than fossil fuels (Fargione et al., 2008; Searchinger et al., 2008). This is controversial, as the allocation of GHG emissions to the management and the use of coproducts can have a large effect on the total carbon footprint of resulting bioenergy products (Whitaker et al., 2010; Davis et al., 2013). The potential consequences of land use change (LUC) to bioenergy on GHG balance through food crop displacement or ‘indirect’ land use change (iLUC) are also an important consideration (Searchinger et al., 2008).»
Milner, S. , Holland, R. A., Lovett, A. , Sunnenberg, G. , Hastings, A. , Smith, P. , Wang, S. and Taylor, G. (2016), Potential impacts on ecosystem services of land use transitions to second‐generation bioenergy crops in GB. GCB Bioenergy, 8: 317-333. https://doi.org/10.1111/gcbb.12263This article incorporates text available under the CC BY 4.0 license. (The CC BY 4.0 licence means that everyone have the right to reuse the text that is quoted here, or other parts of the original article itself, if they credit the authors. More info: https://en.wikipedia.org/wiki/Creative_Commons_license)
^ «While the initial premise regarding bioenergy was that carbon recently captured from the atmosphere into plants would deliver an immediate reduction in GHG emission from fossil fuel use, the reality proved less straightforward. Studies suggested that GHG emission from energy crop production and land-use change might outweigh any CO2 mitigation (Searchinger et al., 2008; Lange, 2011). Nitrous oxide (N2O) production, with its powerful global warming potential (GWP), could be a significant factor in offsetting CO2 gains (Crutzen et al., 2008) as well as possible acidification and eutrophication of the surrounding environment (Kim & Dale, 2005). However, not all biomass feedstocks are equal, and most studies critical of bioenergy production are concerned with biofuels produced from annual food crops at high fertilizer cost, sometimes using land cleared from natural ecosystems or in direct competition with food production (Naik et al., 2010). Dedicated perennial energy crops, produced on existing, lower grade, agricultural land, offer a sustainable alternative with significant savings in greenhouse gas emissions and soil carbon sequestration when produced with appropriate management (Crutzen et al., 2008; Hastings et al., 2008, 2012; Cherubini et al., 2009; Don- dini et al., 2009a; Don et al., 2012; Zatta et al., 2014; Rich- ter et al., 2015).»
McCalmont, J. P., Hastings, A. , McNamara, N. P., Richter, G. M., Robson, P. , Donnison, I. S. and Clifton‐Brown, J. (2017), Environmental costs and benefits of growing Miscanthus for bioenergy in the UK. GCB Bioenergy, 9, page 490. https://doi.org/10.1111/gcbb.12294This article incorporates text available under the CC BY 4.0 license. (The CC BY 4.0 licence means that everyone have the right to reuse the text that is quoted here, or other parts of the original article itself, if they credit the authors. More info: https://en.wikipedia.org/wiki/Creative_Commons_license)
^ «Significant reductions in GHG emissions have been demonstrated in many LCA studies across a range of bioenergy technologies and scales (Thornley et al., 2009, 2015). The most significant reductions have been noted for heat and power cases. However, some other studies (particularly on transport fuels) have indicated the opposite, that is that bioenergy systems can increase GHG emissions (Smith & Searchinger, 2012) or fail to achieve increasingly stringent GHG savings thresholds. A number of factors drive this variability in calculated savings, but we know that where significant reductions are not achieved or wide variability is reported there is often associated data uncertainty or variations in the LCA methodology applied (Rowe et al., 2011). For example, data uncertainty in soil carbon stock change following LUC has been shown to significantly influence the GHG intensity of biofuel production pathways (Fig. 3), whilst the shorter term radiative forcing impact of black carbon particles from the combustion of biomass and biofuels also represents significant data uncertainty (Bond et al., 2013).»
Whitaker, J. , Field, J. L., Bernacchi, C. J., Cerri, C. E., Ceulemans, R. , Davies, C. A., DeLucia, E. H., Donnison, I. S., McCalmont, J. P., Paustian, K. , Rowe, R. L., Smith, P. , Thornley, P. and McNamara, N. P. (2018), Consensus, uncertainties and challenges for perennial bioenergy crops and land use. GCB Bioenergy, 10: 150-164. https://doi.org/10.1111/gcbb.12488This article incorporates text available under the CC BY 4.0 license. (The CC BY 4.0 licence means that everyone have the right to reuse the text that is quoted here, or other parts of the original article itself, if they credit the authors. More info: https://en.wikipedia.org/wiki/Creative_Commons_license)
^ «A life‐cycle perspective of the relative contributions and variability of soil carbon stock change and nitrogen‐related emissions to the net GHG intensity (g CO2‐eq MJ−1) [gram CO2-equivalents per megajoule] of biofuel production via select production pathways (feedstock/prior land‐use/fertilizer/conversion type). Positive and negative contributions to life‐cycle GHG emissions are plotted sequentially and summed as the net GHG intensity for each biofuel scenario, relative to the GHG intensity of conventional gasoline (brown line) and the 50% and 60% GHG savings thresholds (US Renewable Fuel Standard and Council Directive 2015/1513); orange and red lines, respectively. Default life‐cycle GHG source estimates are taken from Wang et al. (2012) and Dunn et al. (2013); direct N2O emissions from Fig. 1; and soil carbon stock change (0–100 cm depth) from Qin et al. (2016). See Appendix S1 for detailed methods.»
Whitaker, J. , Field, J. L., Bernacchi, C. J., Cerri, C. E., Ceulemans, R. , Davies, C. A., DeLucia, E. H., Donnison, I. S., McCalmont, J. P., Paustian, K. , Rowe, R. L., Smith, P. , Thornley, P. and McNamara, N. P. (2018), Consensus, uncertainties and challenges for perennial bioenergy crops and land use. GCB Bioenergy, 10: 150-164. https://doi.org/10.1111/gcbb.12488This article incorporates text available under the CC BY 4.0 license. (The CC BY 4.0 licence means that everyone have the right to reuse the text that is quoted here, or other parts of the original article itself, if they credit the authors. More info: https://en.wikipedia.org/wiki/Creative_Commons_license)
^ «Whilst these values represent the extremes, they demonstrate that site selection for bioenergy crop cultivation can make the difference between large GHG savings or losses, shifting life‐cycle GHG [green house gas] emissions above or below mandated thresholds. Reducing uncertainties in ∆C [carbon increase or decrease] following LUC [land use change] is therefore more important than refining N2O [nitrous oxide] emission estimates (Berhongaray et al., 2017). Knowledge on initial soil carbon stocks could improve GHG savings achieved through targeted deployment of perennial bioenergy crops on low carbon soils (see section 2). […] The assumption that annual cropland provides greater potential for soil carbon sequestration than grassland appears to be over‐simplistic, but there is an opportunity to improve predictions of soil carbon sequestration potential using information on the initial soil carbon stock as a stronger predictor of ∆C [change in carbon amount] than prior land use.»
Whitaker, J. , Field, J. L., Bernacchi, C. J., Cerri, C. E., Ceulemans, R. , Davies, C. A., DeLucia, E. H., Donnison, I. S., McCalmont, J. P., Paustian, K. , Rowe, R. L., Smith, P. , Thornley, P. and McNamara, N. P. (2018), Consensus, uncertainties and challenges for perennial bioenergy crops and land use. GCB Bioenergy, 10: 150-164. https://doi.org/10.1111/gcbb.12488This article incorporates text available under the CC BY 4.0 license. (The CC BY 4.0 licence means that everyone have the right to reuse the text that is quoted here, or other parts of the original article itself, if they credit the authors. More info: https://en.wikipedia.org/wiki/Creative_Commons_license)
^ «Fig. 3 confirmed either no change or a gain of SOC [soil organic carbon] (positive) through planting Miscanthus on arable land across England and Wales and only a loss of SOC (negative) in parts of Scotland. The total annual SOC change across GB in the transition from arable to Miscanthus if all nonconstrained land was planted with would be 3.3 Tg C yr−1 [3.3 million tonnes carbon per year]. The mean changes for SOC for the different land uses were all positive when histosols were excluded, with improved grasslands yielding the highest Mg C ha−1 yr−1 [tonnes carbon per hectare per year] at 1.49, followed by arable lands at 1.28 and forest at 1. Separating this SOC change by original land use (Fig. 4) reveals that there are large regions of improved grasslands which, if planted with bioenergy crops, are predicted to result in an increase in SOC. A similar result was found when considering the transition from arable land; however for central eastern England, there was a predicted neutral effect on SOC. Scotland, however, is predicted to have a decrease for all land uses, particularly for woodland due mainly to higher SOC and lower Miscanthus yields and hence less input.»
Milner, S. , Holland, R. A., Lovett, A. , Sunnenberg, G. , Hastings, A. , Smith, P. , Wang, S. and Taylor, G. (2016), Potential impacts on ecosystem services of land use transitions to second‐generation bioenergy crops in GB. GCB Bioenergy, 8: 317-333. https://doi.org/10.1111/gcbb.12263This article incorporates text available under the CC BY 4.0 license. (The CC BY 4.0 licence means that everyone have the right to reuse the text that is quoted here, or other parts of the original article itself, if they credit the authors. More info: https://en.wikipedia.org/wiki/Creative_Commons_license)
^ Eartha Jane Melzer (January 26, 2010). "Proposed biomass plant: Better than coal?". The Michigan Messenger. Archived from the original on 2010-02-05.
^ Zhang, J.; Smith, K. R. (2007). "Household Air Pollution from Coal and Biomass Fuels in China: Measurements, Health Impacts, and Interventions". Environmental Health Perspectives. 115 (6): 848–855. doi:10.1289/ehp.9479. PMC 1892127. PMID 17589590.
^ "Announcement". Archives of Virology. 130 (1–2): 225. 1993. doi:10.1007/BF01319012.
^ Gustafsson, O.; Krusa, M.; Zencak, Z.; Sheesley, R. J.; Granat, L.; Engstrom, E.; Praveen, P. S.; Rao, P. S. P.; et al. (2009). "Brown Clouds over South Asia: Biomass or Fossil Fuel Combustion?". Science. 323 (5913): 495–8. Bibcode:2009Sci...323..495G. doi:10.1126/science.1164857. PMID 19164746.
^ Springsteen, Bruce; Christofk, Tom; Eubanks, Steve; Mason, Tad; Clavin, Chris; Storey, Brett (2011). "Emission Reductions from Woody Biomass Waste for Energy as an Alternative to Open Burning". Journal of the Air & Waste Management Association. 61 (1): 6. doi:10.3155/1047-3289.61.1.63.
External links
![]() |
Look up biomass in Wiktionary, the free dictionary. |
![]() |
Wikimedia Commons has media related to Biomass. |
- Biomass interactive by PBL Netherlands Environmental Assessment Agency
- Forest, Nature and Biomass
- GA Mansoori, N Enayati, LB Agyarko (2016), Energy: Sources, Utilization, Legislation, Sustainability, Illinois as Model State, World Sci. Pub. Co.,
ISBN 978-981-4704-00-7
- Solid biomass EurObserv'ER barometer – 2012 PDF
lis,y,2CUQp 3O,J0I47X5hMMrvcjXsjitNUnosU3